A superfluid is a state of matter in which matter behaves as if it were fluid with no viscosity. The substance, which appears to be a normal liquid, flows past any surface without friction, allowing it to continue to circulate over obstructions and through pores in containers that hold it, subject only to its own inertia.
We can learn a lot by studying the microscopic and macroscopic changes that occur in material as it transitions from one phase to another, such as ice to water to steam. A new Australian study looks at systems that transition from ‘normal’ fluid to a quantum state known as a superfluid, which can flow with zero friction, with an eye toward future superfluid-based quantum technologies like ultra-low energy electronics. We can learn a lot by studying the microscopic and macroscopic changes that occur in material as it transitions from one phase to another, such as ice to water to steam.
While these phase transitions in water are well understood, much less is known about the dynamics that occur when a system transitions from a normal fluid to a superfluid, which can flow with zero friction, i.e. without losing any energy.
A new study examines systems transitioning from ‘normal’ fluid to a quantum state known as a superfluid, which can flow with zero friction, with a view to future, superfluid-based, quantum technologies, such as ultra-low energy electronics.
A new Swinburne study that observed the transition of an atomic gas from normal fluid to superfluid provides new insights into the formation of these remarkable states, with implications for future superfluid-based quantum technologies like ultra-low energy electronics. The superfluid formation was observed to involve a number of different timescales, each associated with a different dynamical process that occurs when the phase boundary is crossed.
Understanding Dynamic Transitions, towards Future Technologies
Phase transitions are difficult to understand theoretically because they are nonequilibrium, dynamic process within these fascinating and potentially useful states of matter.
In many-body quantum systems, such non-equilibrium phenomena involve a complex interplay of correlations spanning vastly different spatiotemporal scales. The ultrashort timescales can make access to the full dynamics of most materials impossible.
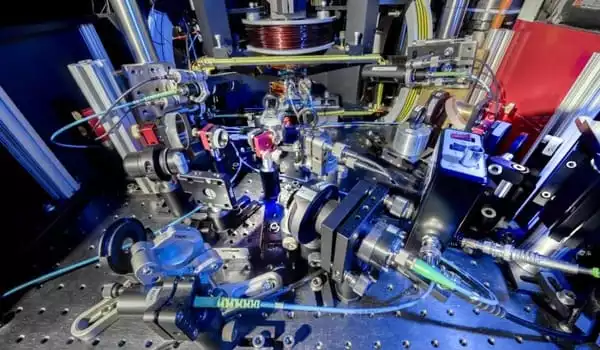
Superfluidity is the property of a fluid that has no viscosity and thus flows with no loss of kinetic energy. When a superfluid is stirred, vortices form that continues to rotate indefinitely. When two isotopes of helium are liquefied by cooling to cryogenic temperatures, superfluidity occurs. It is also a property of various other exotic states of matter that is thought to exist in astrophysics, high-energy physics, and quantum gravity theories. Lev Landau and Isaak Khalatnikov, two Soviet theoretical physicists, developed the theory of superfluidity.
Future quantum-state technologies, such as superfluids or superconductors, will need to be ‘switched’ (on/off), so understanding how systems evolve after switching answers fundamental questions such as how fast such devices can operate. The correlated motion of many microscopic constituents within a large collection of quantum-mechanical particles is required to form a superfluid.
“However, dilute gases of ultracold atoms allow measurements of real-time dynamics on accessible timescales,” says lead author Dr. Paul Dyke (Swinburne). “We use an ultracold gas of strongly interacting fermionic atoms (ie, a Fermi gas) to investigate how the correlations required to form a superfluid build up after the interactions are suddenly quenched. This throws the system out of balance.”
“We can resolve the different timescales involved for the various correlations to build up by measuring the subsequent dynamics as the system returns to equilibrium. These timescales are determined by the length scales, with short-range correlations and pair formation developing quickly while the overall momentum distribution can take several orders of magnitude longer to reach equilibrium.”
The new experiment showed that:
- Depending on the speed of the quench, the formation and condensation of fermion pairs can occur on very different timescales.
- The contact parameter is observed to respond very quickly to changes in interaction strength, indicating that short-range correlations evolve much faster than long-range correlations required to form a Bose-Einstein condensate of atom pairs.
The contact parameter quantifies the likelihood of finding two atoms very close to each other, and it is greatly increased when atoms form pairs.