By chance, the famous British researcher Michael Faraday made an amazing discovery in 1842: Even though the temperature is well below zero degrees, a thin layer of water forms on the surface of ice. Despite the fact that the temperature is below the melting point of ice, the ice’s surface has melted. This liquid layer on ice crystals is also responsible for snowballs sticking together.
This “surface melting” could not be scientifically confirmed under controlled laboratory conditions until about 140 years later, in 1985. Surface melting has now been demonstrated in a wide range of crystalline materials and is well understood scientifically: A liquid layer only a few nanometers thick forms on the surface of the otherwise solid material several degrees below the actual melting point.
Because the surface properties of materials play an important role in their use as catalysts, sensors, battery electrodes, and other applications, surface melting is not only fundamental but also important in terms of technical applications.
It should be noted that this procedure has nothing to do with the effect of, say, taking an ice cube out of the freezer and exposing it to room temperature. Under such conditions, an ice cube melts on its surface first because the surface is significantly warmer than the ice cube’s interior.
The researchers were able to demonstrate the process of surface melting in such a colloidal glass because the particles near the surface move much faster compared to the solid below. At first glance, such behavior is not entirely unexpected, since the particle density at the surface is lower than in the underlying bulk material.
Surface melting detected in glass
In crystals with periodically arranged atoms, the thin liquid layer on the surface is typically detected by scattering experiments, which are very sensitive to the presence of atomic order. Since liquids are not arranged in a regular pattern, such techniques can therefore clearly resolve the appearance of a thin liquid film on top of the solid.
This approach, however, does not work for glasses (i.e. disordered, amorphous materials) because there is no difference in the atomic order between the solid and the liquid. Therefore, surface melting of glasses has remained rather unexplored with experiments.
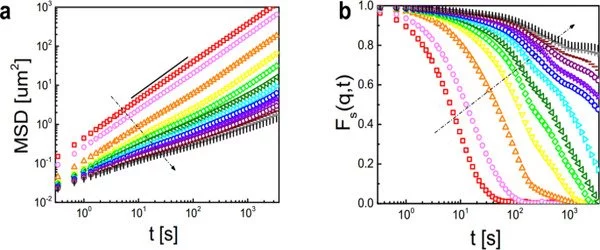
To overcome the above-mentioned difficulties, Clemens Bechinger, a physics professor at the University of Konstanz, and his colleague Li Tian used a trick: instead of studying atomic glass, they produced a disordered material made of microscopic glass spheres known as colloids. In contrast to atoms, these particles are about 10,000 times larger and can be observed directly under a microscope.
The researchers were able to demonstrate the process of surface melting in such a colloidal glass because the particles near the surface move much faster compared to the solid below. At first glance, such behavior is not entirely unexpected, since the particle density at the surface is lower than in the underlying bulk material. Therefore, particles close to the surface have more space to move past each other, which makes them faster.
A surprising discovery
Clemens Bechinger and Li Tian were surprised to discover that even far below the surface, where particle density has reached bulk levels, particle mobility is still significantly higher than in bulk material.
The microscope images show that this previously unknown layer is up to 30 particle diameters thick and extends in a streak-like pattern from the surface into the deeper regions of the solid. “Bechinger explains that this layer, which extends deep into the material, has interesting material properties because it combines liquid and solid features.”
As a result, the properties of thin, disordered films are highly dependent on their thickness. This property is already being used in their use as thin ionic conductors in batteries, which have been shown to have significantly higher ionic conductivity than thick films. However, with the new insights gained from the experiments, this behavior can now be quantified and thus optimized for technical applications.