Chemists at the DOE’s Brookhaven National Laboratory have developed a new theoretical framework for better forecasting catalyst activity. These clusters of atoms reduce the amount of energy required for numerous chemical reactions. The study demonstrates how temperature and pressure can affect a catalyst’s structure, efficiency, and even the goods it produces. The findings were published in the journal Chem Catalysis.
“Our results highlight the significant impact the reaction environment can have on catalytic performance,” said Ping Liu, a theorist in Brookhaven Lab’s Chemistry Division who is also an adjunct professor at Stony Brook University (SBU) and oversaw the research. “We show that these catalyst-environment interactions can be used to tune the efficiency and selectivity of catalysts, which could point to new ways to design better catalysts.”
The researchers modeled catalysts that assist hydrogen (H2) transform carbon dioxide (CO2), a greenhouse gas, into a variety of other compounds, including methanol. The catalysts were constructed of palladium (Pd) combined with other metals, such as zinc (Zn) or silver (Ag), which scientists had previously proved to be effective for the “CO2 hydrogenation” reaction.
They were inspired by a significant divergence in prior study on this reaction. Previously reported tests showed that metallic palladium preferentially created formic acid (HCOOH). However, theoretical calculations indicated that methanol (H3COH) should be the most energetically favorable product.
Our results highlight the significant impact the reaction environment can have on catalytic performance. We show that these catalyst-environment interactions can be used to tune the efficiency and selectivity of catalysts, which could point to new ways to design better catalysts.
Ping Liu
“This contradiction between theory and experiment made us wonder why there are differences. What are we missing?” Liu asked. Hong Zhang, Liu’s graduate student at SBU and the first author on the paper, designed a way to find out by modeling what happens during the reaction.
“We developed a framework based on density function theory and kinetic modeling to capture the dynamic behavior and structure of the catalyst under operational reaction conditions,” Zhang told me.
Calculations using density function theory reveal the most likely atomic configurations and interactions. Kinetic modeling depicts how reactants transition from one step of the reaction to the next via a sequence of intermediates. The goal of this technique is to bridge the gap between studies of catalysts in their cleanest, freshly created state and studies that look at catalysts after a reaction is complete.
“The reality is that a catalyst often undergoes significant structural changes or phase transitions in the reaction environment,” Liu told me. “But those reaction-driven dynamics are difficult to capture experimentally at the atomic level, even using the amazing characterization tools we have for studying catalysts in real time.”
She stated that the new modeling approach, together with experimental characterisation methods, will provide the precise understanding of catalytic mechanisms required to guide future catalyst designs.
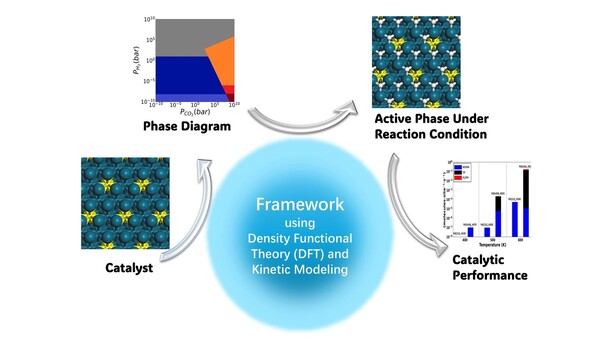
The modeling framework
Zhang explained how the framework works.
“We start with modeling the as-prepared catalyst – in this case, zinc deposited onto the palladium surface,” he said. “Experimentally, after preparing the catalyst, scientists expose the sample to the mixture of hydrogen and carbon dioxide. We do the same thing using modeling,” Zhang said.
“We consider many representative ‘species’ — reactants and intermediates — that could be present or could form in the particular reaction conditions and have the potential to be stable, as well as how the surface of the catalyst might change.”
The scientists then mapped out the “phase changes” in the catalyst under various carbon dioxide and hydrogen pressures and temperatures. They discovered which circumstances accelerated the reaction and altered the pathway to yield distinct chemicals.
At normal temperature, they discovered that the catalytic surface was essentially covered in hydrogen, preventing the process from starting. Raising the temperature created hydrogen vacancies, allowing carbon dioxide to reach the active palladium sites. That access triggered the conversion of carbon dioxide and hydrogen into formic acid.
“Going to higher temperatures, we saw even more of a decrease in hydrogen coverage,” Liu said.
More hydrogen vacancies further increased the conversion of carbon dioxide.
“This was expected since most reactions proceed faster at higher temperatures,” Liu explained. “But we also saw a change in selectivity. The reaction went from producing formic acid to producing more and more carbon monoxide and methanol,” she said.
The change in selectivity was somewhat unexpected, but it explained the prior discrepancy between theory and experiment.
“We found that changing the temperature actually changes the active sites of the catalyst, from single hydrogen vacancies to dimers or trimers — pairs or triplets — of missing hydrogens,” Liu said. “Those larger hydrogen vacancies change the catalyst’s selectivity to favor methanol production,” she said.
The scientists then confirmed and proved that the framework is suitable for simulating various catalysts. They tested it on pure palladium, a bulk palladium and zinc alloy in which the zinc is not limited to the subsurface layer, and a bulk palladium and silver alloy.
“We simply computed the surface diagram of these catalysts using the experimental settings that others have investigated. We can easily anticipate selectivity based on surface coverage under these conditions,” Liu stated. “In all three cases, the framework we developed can accurately describe the experimentally observed selectivity with significantly reduced computing cost.”
This work, she added, has improved the interplay between theoretical and experimental approaches to understanding catalyst architectures and mechanisms, as well as how reaction circumstances can be used to control catalytic activity.
“This whole framework goes way beyond the CO2 hydrogenation reaction and the palladium-based catalysts,” Liu told me. “It allows you to obtain a better knowledge of catalysts’ active sites and how they perform under operating settings. This will aid in the establishment of a more precise structure-catalysis link, which is critical for the creation of active and selective catalysts.