Photosynthesis is a complex process that converts light energy into chemical energy in the form of adenosine triphosphate (ATP) and reduced nicotinamide adenine dinucleotide phosphate (NADPH) in plants, algae, and some microorganisms. The first step in photosynthesis is to absorb light energy through light-absorbing pigments like as chlorophyll and other accessory pigments.
The energy transfer between photosynthetic light-harvesting proteins has been quantified by chemists. They discovered that a disorganised arrangement of light-harvesting proteins improves energy transduction efficiency.
When photosynthetic cells absorb sunlight, energy packets known as photons jump amongst a sequence of light-harvesting proteins until they reach the photosynthetic reaction centre. Cells turn the energy into electrons, which are then used to produce sugar molecules. This energy transfer occurs at an exceptionally high efficiency: nearly every photon of light received generates an electron, a phenomenon known as near-unity quantum efficiency.
Long-distance energy transduction is required for that antenna to function. Our key finding is that the disordered organisation of the light-harvesting proteins improves the efficiency of that long-distance energy transduction.
Gabriela Schlau-Cohen
A new MIT study gives a possible explanation for how proteins in the light-harvesting complex, often known as the antenna, attain such great efficiency. The researchers were able to measure the energy transfer between light-harvesting proteins for the first time, leading them to uncover that the disorganised organisation of these proteins increases the efficiency of energy transduction.
“Long-distance energy transduction is required for that antenna to function. Our key finding is that the disordered organisation of the light-harvesting proteins improves the efficiency of that long-distance energy transduction,” explains Gabriela Schlau-Cohen, senior author of the current study and an associate professor of chemistry at MIT.
For decades, researchers have studied the photosynthetic process to better understand its efficiency, and they have made tremendous progress in unravelling its molecular underpinnings. These discoveries have been aided by advancements in scientific techniques such as X-ray crystallography and spectroscopy.
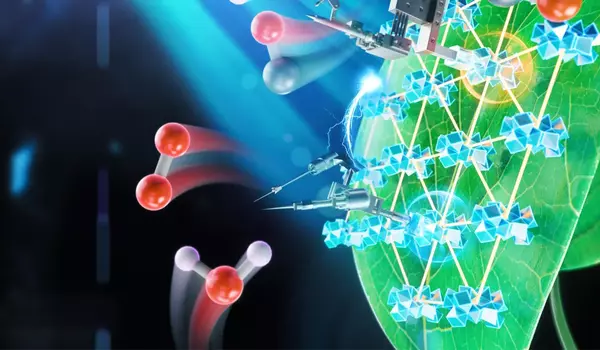
Energy capture
The MIT researchers focused on purple bacteria for this work because they are widely found in oxygen-poor aquatic habitats and are commonly utilised as a model for investigations of photosynthetic light-harvesting.
Captured photons move through light-harvesting complexes made up of proteins and light-absorbing pigments like chlorophyll within these cells. Scientists were able to investigate how energy transfers within a single one of these proteins using ultrafast spectroscopy, a technique that employs extremely brief laser pulses to study events that occur on timescales ranging from femtoseconds to nanoseconds. However, examining how energy transfers between these proteins has been far more difficult because it requires precisely placing many proteins.
The MIT researchers built synthetic nanoscale membranes with a composition comparable to that of naturally occurring cell membranes to construct an experimental setup where they could monitor how energy transfers between two proteins. They were able to adjust the distance between two proteins implanted within the discs by altering the size of these membranes, known as nanodiscs.
The researchers used nanodiscs to insert two copies of the major light-harvesting protein found in purple bacteria, known as LH2 and LH3. LH2 is the protein that is present under normal lighting settings, whereas LH3 is a variation that is typically expressed only under low lighting conditions.
Using the cryo-electron microscope at the MIT.nano facility, the researchers could image their membrane-embedded proteins and show that they were positioned at distances similar to those seen in the native membrane. They were also able to measure the distances between the light-harvesting proteins, which were on the scale of 2.5 to 3 nanometers.
Disordered is better
Because LH2 and LH3 absorb slightly different wavelengths of light, it is possible to use ultrafast spectroscopy to observe the energy transfer between them. For proteins spaced closely together, the researchers found that it takes about 6 picoseconds for a photon of energy to travel between them. For proteins farther apart, the transfer takes up to 15 picoseconds.
Because the longer the voyage, the more energy is lost during the transfer, faster speed leads to more efficient energy transfer.
“When a photon is absorbed, there is only so much time before that energy is lost through unwanted processes like nonradiative decay, so the faster it can be converted, the more efficient it will be,” Schlau-Cohen explains.
The researchers also discovered that proteins ordered in a lattice form transferred energy less efficiently than proteins assembled in randomly organised patterns, as they do in real cells.
“Ordered organisation is actually less efficient than biology’s disordered organisation, which we find really interesting because biology is disordered. This finding suggests that this may not be an unavoidable side effect of biology, but that organisms may have evolved to take advantage of it,” Schlau-Cohen says.
After establishing the capacity to quantify inter-protein energy transfer, the researchers intend to investigate energy transfer between additional proteins, such as the transfer between antenna proteins and reaction centre proteins. They also intend to investigate the transfer of energy across antenna proteins found in organisms other than purple bacteria, such as green plants.