Matter is common in our own backyard, but antimatter is uncommon. In fact, except for high-energy events that produce equal amounts of matter and antimatter — such as electron-positron pairs — there is no antimatter anywhere we look. Our Milky Way’s planets, stars, gas, dust, and other objects are all constituted of matter, not antimatter. All of the galaxies we see beyond our own are composed of matter, not antimatter. Galaxy clusters and the large-scale cosmic web indicate that everything is constituted of matter rather than antimatter. All of the usual material, the stuff of the Standard Model, is all “matter” in our Universe, with almost no antimatter.
Most of the time, we ask the big baryogenesis question: how did the Universe come to be made of matter rather than antimatter? But, before we get there, are we positive that the Universe is formed of matter and that there isn’t a vast collection of antimatter somewhere out there? Tim Thompson wants to know, so he asks:
“How do we know it’s primarily one or the other?” Can we discern if a system is matter or antimatter from a distance? For example, what is it that tells us about a galaxy millions of light years away that we can only view through photons emitted?”
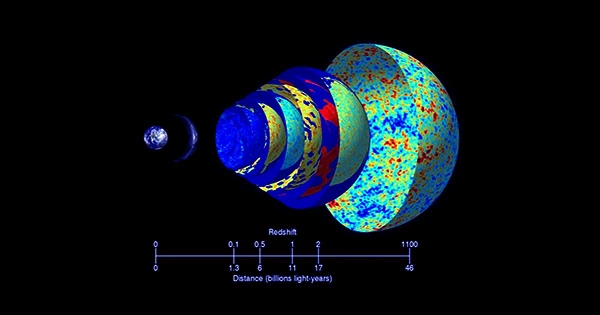
It’s an excellent question. Fortunately, astronomy and astrophysics have the answer.
When matter and antimatter collide in the Universe, they annihilate, and the resulting signal is incredibly particular. When a particle of matter collides with its antimatter counterpart, two photons with equal energy and opposite momenta are often produced (in the collision’s center-of-momentum frame of reference). An electron colliding with a positron, for example, creates two photons with exactly 511,000 electron volts of energy each: the energy equivalent of the mass of the colliding particles, according to Einstein’s E = mc2.
We may observe these annihilation signals anywhere in space, allowing us to pinpoint where matter and antimatter collide. If there was:
- planets
- stars
- galaxies
- clusters of galaxies
- even intergalactic space,
We’d detect evidence of those high-energy photons from annihilation at the interface where some were matter and others were antimatter. The fact that we observe such photons, albeit infrequently and only in specific locations (mainly compatible with emissions from pulsars and active black holes), allows us to impose enormous limitations on what fraction of the Universe can be formed of antimatter on a number of scales.
Within a galaxy, you must know that stars are not isolated objects, but rather have extended structures surrounding them, such as planets and moons, zodiacal dust on a plane, a Kuiper-like belt and a scattered disk, and an Oort-like cloud spanning a light-year in any direction. Another star/star system will pass within one light-year or fewer of any given star about once every million years – remember, we’re already living in a Universe that’s 13.8 billion years old (or, to put it another way, 13,800 million years old). That means that over the course of a star’s lifespan, it should interact with thousands of other stars/star systems in our galaxy.
If there were any antimatter stars, complete with antimatter planets, antimatter moons, and antimatter bodies in their disk and surrounding cloud, there would be a massive release of energy whenever the antimatter from that system interacted with the matter from our galaxy’s remaining stars. The absence of high-energy emissions, like as gamma-ray bursts, from within our galaxy strongly suggests that there are no antimatter stars within our galaxy. The absence of antimatter in adjacent galaxies substantially limits the quantity of antimatter that could exist within them.
This problem can also be scaled up to bigger cosmic proportions. There have been several sightings of galaxies traveling through galaxy groupings and galaxy clusters, some of which move at breakneck speed. We discover a lot of evidence for stars and gas in the intracluster medium (the space between the galaxies in the cluster), and this gas interacts with the galaxies that move through it. In and around these galaxies, we can witness the impacts of gas stripping, tidal disturbance, and star formation. However, there is no evidence of matter-antimatter annihilation.
In other words, if any of the galaxies within a galaxy group or a galaxy cluster were formed of antimatter, we would see the consequences of matter-antimatter annihilation where these antimatter galaxies interact with the rest of the group or cluster. The fact that we’ve observed dozens upon thousands of galaxy groups and clusters in the Universe and have never encountered a signal consistent with this form of matter-antimatter annihilation substantially limits the amount of antimatter that could exist.
- On the grandest cosmic sizes of all, we can examine three distinct types of systems.
- We can look at clusters of galaxies that are colliding and merging.
- We may look at individual galaxy clusters that are colliding.
- We can also look at the large-scale cosmic web, where massive structures — clusters of galaxies — can congregate in filaments that span billions of light-years.
We find evidence for all of the intricate physics we would expect to see if everything in the system is formed of the same sort of matter: either 100% matter or 100% antimatter.
Where collisions occur, we witness gas heating up and generating X-rays. As the “normal” stuff encounters pull, heating, and the birth of new stars, we detect signs of this material separating from dark matter, but the dark matter simply travels right through itself and the normal stuff unimpeded. We notice the polarization of the emitted light revolving (Faraday rotation), which is consistent with the presence of magnetic fields on galactic scales. And, once again, we see a complete lack of matter-antimatter annihilation, demonstrating that there are no “matter” and “antimatter” regions in touch with one another.
It’s also feasible that our Universe was created with a network of topological flaws, such as:
We might have a discontinuity where matter dominates on one side of the defect and antimatter dominates on the other side of the defect in dimensional defects such as cosmic strings, dimensional defects such as domain walls, and dimensional defects such as cosmic textures.
Unfortunately for these possibilities, the large-scale clustering evidence in the Universe, as well as extensive investigations of the cosmic microwave background, have all ruled them out with extreme certainty.
There are several theoretical methods that may be proposed to produce split zones in space, where one part contains matter and the other includes antimatter, but all of them share at least one of the following two characteristics:
- They cause a break in the Universe’s clustering data, which would have been visible in galaxy surveys.
- They form an interface between matter and antimatter areas, resulting in lines, sheets, or larger regions where matter and antimatter annihilate.
Because these features are not observed, we can safely conclude that our Universe is, for all intents and purposes, 100% matter with barely a trace of antimatter.
But suppose you needed a completely different line of data to examine in order to establish the amount of matter in the Universe. Would such a thing exist in the absence of stars, galaxies, clusters of galaxies, and the gamma-ray sky?
Yes, we have an abundance of light elements synthesized during the early stages (the first few minutes) of the hot Big Bang, which were created during the early phases of nucleosynthesis.
Because the wavelength of each light wave defines its energy, and the Universe expands through time, the wavelength of each photon gets stretched as time passes. However, if we extrapolate backward, we find that the wavelength of each photon was shorter — more compressed — in the past, implying that the further back in time we look, the hotter the Universe was in its early phases. At some point in time, the Universe became so hot that neutral atoms could not form because there were not enough photons with enough energy to prohibit electrons from stably binding to the atomic nuclei that were present. But if we want, we can go back even more.
We can travel back in time to a time when the Universe was so hot that even atomic nuclei couldn’t join together. Every time they tried, a photon shattered the individual protons and neutrons, preventing them from combining to form heavier atoms. Only once the Universe has cooled below a certain critical level — approximately 3 to 4 minutes after the start of the hot Big Bang — can we begin building atomic nuclei heavier than a single, simple proton.
Once that moment occurs, we can use nuclear physics to construct the Universe’s lightest elements.
Surprisingly, the ratio of light elements and isotopes that we receive out, including:
Hydrogen (a single proton), deuterium (a proton plus a neutron), helium-3 (two protons plus a neutron), helium-4 (two protons and two neutrons), and lithium-7 (four protons and three neutrons) are the elements that make up the periodic table.
The ratio of photons to the total number of protons and neutrons combined is the sole metric that matters. Observations from the most pristine clouds of gas we can locate, as well as the imprint in the cosmic microwave background, yield the same result: there is approximately one proton or neutron for every 1.6 billion photons in the Universe. There was more matter than antimatter even in the very early moments of the scorching Big Bang.
On the one hand, this is a positive development. If the Universe contained equal amounts of matter and antimatter, practically all of it would have annihilated. At the moment, the Universe contains fewer than one particle of matter or antimatter every cubic kilometer.
However, the Universe is nearly a billion times denser than that, and virtually all of what remains is matter, not antimatter. However, the only technique we know of converting energy into mass or mass into energy always produces the same result: the number of matter particles minus the number of antimatter particles is always a constant.
Something other than what the Standard Model predicts has to be happening with the particles in the Universe to generate the Universe as we see it today. To approach the problem scientifically, we must extrapolate back to the earliest state of the hot Big Bang, where particles and antiparticles of all types could easily be created at the highest energies, and see what it would take for the Universe to create a matter-antimatter asymmetry where none previously existed.
This is why we are so concerned about the topic of baryogenesis, or how matter became more abundant than antimatter in the Universe. Yes, there are certain general rules for creating one from an originally symmetric state, as demonstrated by Soviet scientist Andrei Sakharov in 1967. All you have to do is meet three criteria known as the Sakharov conditions:
- The Universe’s temperature must be out of balance.
- There must be examples of both C-symmetry and CP-symmetry violations in the Universe.
- Interactions that violate the conservation of baryon number must be permitted throughout the Universe.
While we don’t know exactly how the Universe came to have more matter than antimatter, we do know that it was an essential step in allowing our Universe, and the things and animals within it, to exist as they do. Many experiments from around the world are continually exploring matter and antimatter at subatomic scales, looking for evidence of baryon-number violation as well as additional C-symmetry and CP-symmetry violating interactions.
Observations, on the other hand, completely rule out a Universe with more matter than antimatter. We may not have discovered the “tree of life” that enabled our own existence, but based on what we know so far about physics, we may be confident we’re looking in the right forest.